Our concepts of how the two and a half pounds of flabby flesh between our ears accomplish learning date to Ivan Pavlov’s classic experiments, where he found that dogs could learn to salivate at the sound of a bell. In 1949 psychologist Donald Hebb adapted Pavlov’s “associative learning rule” to explain how brain cells might acquire knowledge. Hebb proposed that when two neurons fire together, sending off impulses simultaneously, the connections between them—the synapses—grow stronger. When this happens, learning has taken place. In the dogs’ case, it would mean the brain now knows that the sound of a bell is followed immediately by the presence of food. This idea gave rise to an oft-quoted axiom: “Synapses that fire together wire together.”
The theory proved sound, and the molecular details of how synapses change during learning have been described in depth. But not everything we remember results from reward or punishment, and in fact, most experiences are forgotten. Even when synapses do fire together, they sometimes do not wire together. What we retain depends on our emotional response to an experience, how novel it is, where and when the event occurred and our level of attention and motivation during the event, and we process these thoughts and feelings while asleep. A narrow focus on the synapse has given us a mere stick-figure conception of how learning and the memories it engenders work.
It turns out that strengthening a synapse cannot produce a memory on its own, except for the most elementary reflexes in simple circuits. Vast changes throughout the expanse of the brain are necessary to create a coherent memory. Whether you are recalling last night’s conversation with dinner guests or using an acquired skill such as riding a bike, the activity of millions of neurons in many different regions of your brain must become linked to produce a coherent memory that interweaves emotions, sights, sounds, smells, event sequences and other stored experiences. Because learning encompasses so many elements of our experiences, it must incorporate different cellular mechanisms beyond the changes that occur in synapses. This recognition has led to a search for new ways to understand how information is transmitted, processed and stored in the brain to bring about learning. In the past 10 years neuroscientists have come to realize that the iconic “gray matter” that makes up the brain’s outer surface—familiar from graphic illustrations found everywhere from textbooks to children’s cartoons—is not the only part of the organ involved in the inscription of a permanent record of facts and events for later recall and replay. It turns out that areas below the deeply folded, gray-colored surface also play a pivotal role in learning. In just the past few years a series of studies from my laboratory and others has elucidated these processes, which could point to new ways of treating psychiatric and developmental disorders that occur when learning impairments arise.
If synaptic changes alone do not suffice, what does happen inside your brain when you learn something new? Magnetic resonance imaging methods now enable researchers to see through a person’s skull and examine the brain’s structure. In scrutinizing MRI scans, investigators began to notice differences in the brain structure of individuals with specific highly developed skills. Musicians, for example, have thicker regions of auditory cortex than nonmusicians. At first, researchers presumed that these subtle differences must have predisposed clarinetists and pianists to excel at their given skills. But subsequent research found that learning changes the structure of the brain.
The kind of learning that leads to alterations in brain tissue is not limited to repetitive sensorimotor skills such as playing a musical instrument. Neuroscientist Bogdan Draganski, currently at the University of Lausanne in Switzerland, and his colleagues witnessed increases in the volume of gray matter in medical students’ brains after they studied for an examination. Many different cellular changes could expand gray matter volume, including the birth of new neurons and of nonneuronal cells called glia. Vascular changes and the sprouting and pruning of axons and dendrites that extend from the main body of a neuron could also do the same. Remarkably, physical changes in the brain can happen much faster during learning than might be expected. Yaniv Assaf of Tel Aviv University and his colleagues showed that 16 laps around a race track in a computerized video game were enough to cause changes in new players’ hippocampal brain region. Structural alterations in the hippocampus in these gamers make sense because this brain region is critical for spatial learning for navigation. In other studies, Assaf and, separately, Heidi Johansen-Berg of the University of Oxford were surprised to find changes in unexpected parts of the brain, including regions that have no neurons or synapses—areas known as white matter.
Deep Learning
Consciousness arises from the cerebral cortex, the three-millimeter-thick outer layer of the human brain, so this gray matter layer is where most researchers expected to find learning-induced modifications. But below the surface layer, billions of tightly packed bundles of axons (nerve fibers), much like tightly wound fibers under the leather skin of a baseball, connect neurons in the gray matter into circuits.
These fiber bundles are white because the axons are coated with a fatty substance called myelin, which acts as electrical insulation and boosts the speed of transmission by 50 to 100 times. White matter injury and disease are important areas of research, but until recently little attention had been given in these investigations to a possible role of myelin in information processing and learning.
In the past 10 or so years studies have begun to find differences in white matter in brain scans of experts with a variety of skills, including people with high proficiency in reading and arithmetic. Expert golfers and trained jugglers also show differences in white matter compared with novices, and white matter volume has even been associated with IQ. If information processing and learning arise from the strengthening of synaptic connections between neurons in gray matter, why does learning affect the brain’s subsurface cabling?
A possible answer began to emerge from cellular studies in my lab investigating how synapses—but also other brain areas—change during learning. The reason for looking beyond the synapse was that most of the drugs we have for treating neurological and psychological disorders work by altering synaptic transmission, and there is a pressing need for more effective agents. The present focus on synaptic transmission might cost us opportunities for better treatments for dementia, depression, schizophrenia or post-traumatic stress disorder (PTSD).
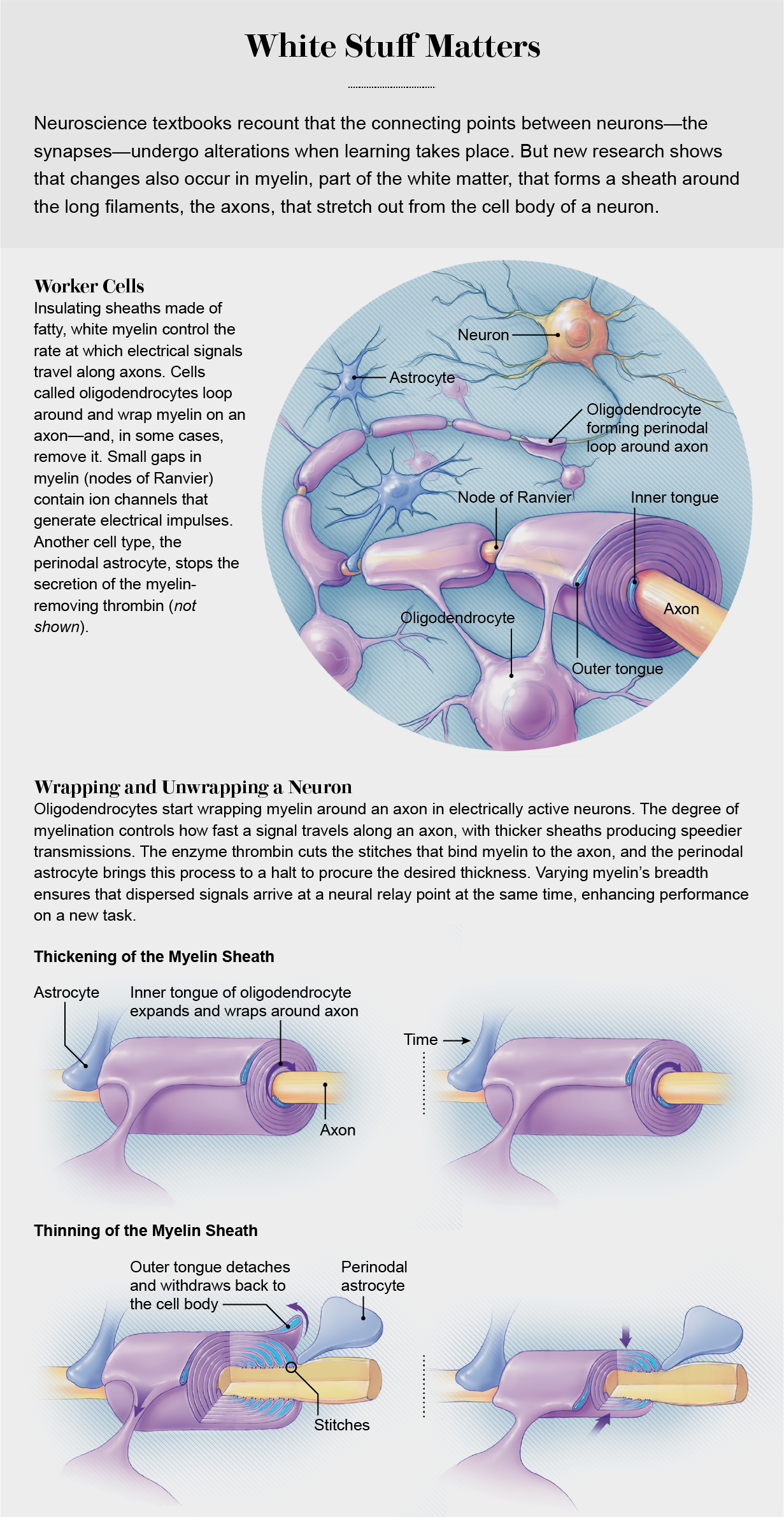
In the early 1990s my lab at the National Institutes of Health and others began to explore the possibility that glia might be able to sense information flowing through neural networks and alter it to improve performance. Experimental evidence that has accumulated since then shows that all types of glial cells respond to neural activity and can modify information transmission in the brain. One of the most surprising of these new findings involves myelin.
Myelin insulation is formed by layers of cell membrane wrapped around axons like electrical tape. In the brain and spinal cord, octopus-shaped glial cells (oligodendrocytes) do the wrapping. In the limbs and trunk, sausage-shaped glial cells (Schwann cells) perform the same task. Many oligodendrocytes grip an axon and wrap layers of myelin around it in segments, like the stacked hands of baseball players gripping a bat to determine which team bats first. The tiny gap between two myelin segments exposes a one-micron section of bare axon where ion channels that generate electrical impulses become concentrated. These spaces, known as the nodes of Ranvier, act like bioelectric repeaters to relay an electrical impulse from node to node down the axon. The speed of impulse transmission increases as more layers of myelin are wrapped around the axon, protecting it better against voltage loss. Also, as a node of Ranvier becomes squeezed more tightly by the adjoining myelin segments, an electrical impulse is initiated more rapidly because it takes less time to charge the smaller amount of nodal membrane to the voltage that triggers ion channels to open and generate an impulse.
Disorders that damage myelin, such as multiple sclerosis and Guillain-Barré syndrome, can cause serious disability because neural impulse transmission fails when the insulation is damaged. But until recently, the idea that myelin might be modified routinely by neural impulses was not widely accepted. And even if myelin structure changed, how and why would this improve performance and learning?
The explanation was hiding in plain sight. It loops back to the old maxim about neurons firing and wiring together. In any complex information or transportation network, the time of arrival at network relay points is critical—think of missing a connection because your flight arrives too late.
How, then, does the transmission speed in every link in the human brain get timed appropriately so that an impulse arrives just when needed? We know that electrical signals shuffle along at the pace of a slow walk in some axons but blaze away at the speed of a race car in others. Signals from two axons that converge on neurons that act as relay points will not arrive together unless the travel time from their input source is optimized to compensate for differences in the lengths of the two axons and the speed at which impulses travel along each link.
Because myelin is the most effective means of speeding impulse transmission, axon myelination promotes optimal information transmission through a network. If oligodendrocytes sense and respond to the information traffic flowing through neural circuits, then myelin formation and the way it adjusts impulse-transmission speed could be controlled by feedback from the axon. But how can myelinating glia detect neural impulses flowing through axons?
Signal Transmission
Over the past two decades our research and that of other labs has succeeded in identifying many neurotransmitters and other signaling molecules that convey to glia the presence of electrical activity in the axon to stimulate myelination. Our experiments have shown that when a neuron fires, neurotransmitters are released not only at synapses but also all along the axon. We found that the “tentacles” of the octopuslike oligodendrocytes probe bare sections of axons in search of neurotransmitters being released from axons firing. When a single tentacle touches an axon that is firing, it forms a “spot weld” contact, which enables communication between the axon and the oligodendrocyte. The oligodendrocyte begins to synthesize myelin at that spot and wrap it around the axon.
When we gave oligodendrocytes in cell culture the choice of myelinating electrically active axons or ones treated with botulinum toxin to prevent the release of neurotransmitters, the oligodendrocytes opted for the electrically active axons over the silent ones by a factor of eight to one. So it may be that as a person learns to play “Für Elise” on the piano, bare axons are wrapped with myelin or the volume of existing sheaths is increased in circuits that are activated repetitively during practice, which speeds information flow through brain networks. New myelin then shows up on an MRI as changes in white matter tracts in parts of the brain that are necessary for musical performance.
Several labs have verified that action potentials, signals coursing the length of axons, stimulate myelination of these exposed areas of neural wiring. In 2014 Michelle Monje’s lab at Stanford University showed that optogenetic stimulation (using lasers to make neurons fire) increased myelination in the mouse brain. That same year William Richardson’s lab at University College London demonstrated that when the formation of new myelin is prevented, mice are slower to learn how to run on a wheel with some of its rungs removed. In studies where they used a confocal microscope to watch myelin form in live zebra fish, researchers in David Lyons’s lab at the University of Edinburgh and in Bruce Appel’s lab at the University of Colorado Denver observed that when the release of small sacs containing neurotransmitters from axons is inhibited, often the first few wraps of myelin slip off, and the oligodendrocyte aborts the entire process.
In 2018, working with our colleagues, including Daisuke Kato and others from various institutions in Japan, we showed how myelin promotes learning by ensuring that various spiking electrical signals traveling along axons arrive at the same time in the motor cortex, the brain region that controls movement. Using genetically modified mice with impaired myelination that had been trained to pull a lever to receive a reward, we found that learning this task increased myelination in the motor cortex.
By using electrodes to record neural impulses, we found that action potentials were less synchronized in the motor cortices of mice with faulty myelination. We then boosted the synchronization of spike arrivals in the motor cortex by using optogenetics to make neurons fire at the appropriate time. The mice with impaired myelination then performed the learned task proficiently. Eventually less invasive forms of brain stimulation may become effective therapy to treat neurological and psychological disorders caused by disrupted myelination.
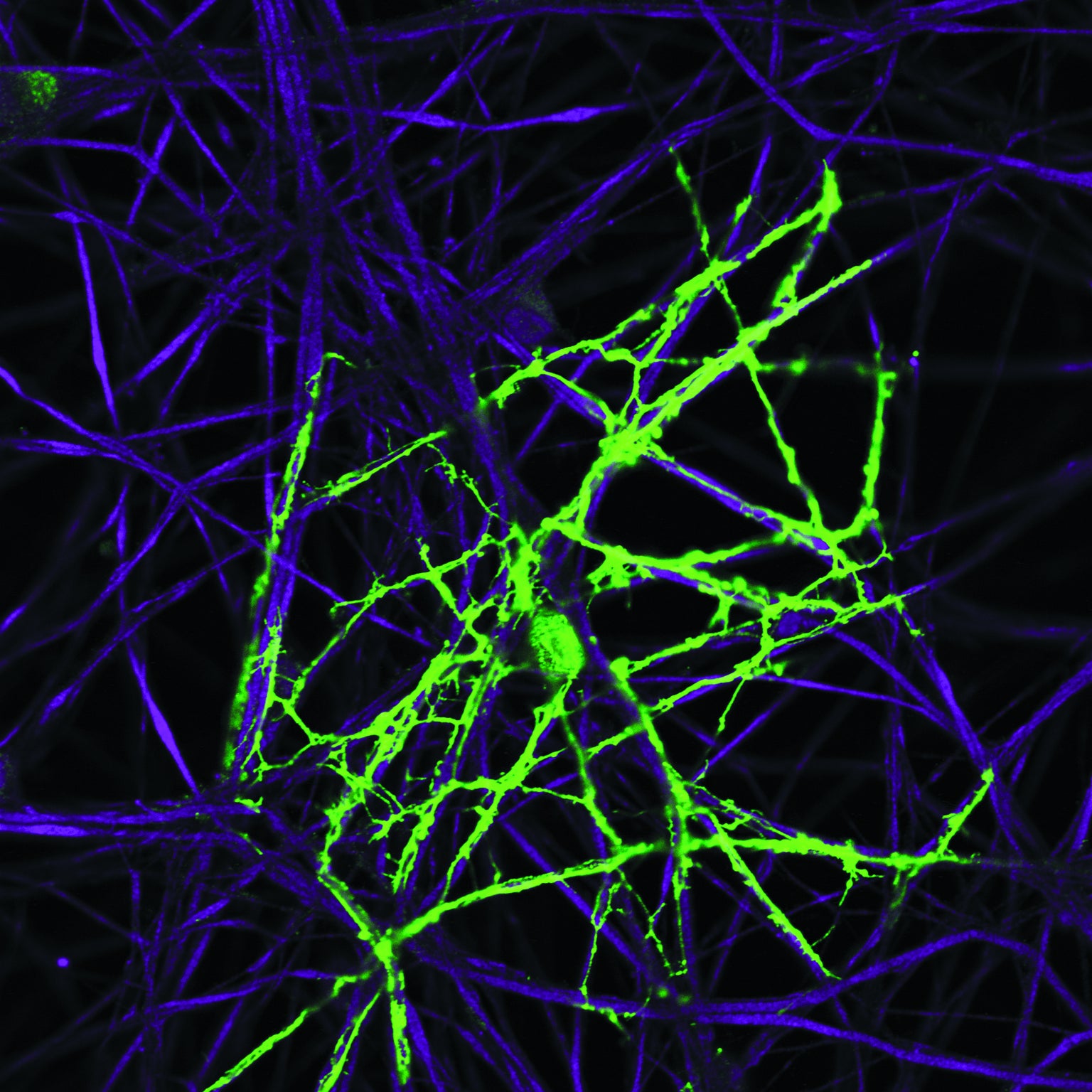
Despite these recent advances, stimulation to increase axon myelination is not always enough to enable new learning, because we cannot synchronize the arrival of spikes at critical relay points in neural networks simply by making the impulses travel as rapidly as possible. There must also be a way to slow the speed of impulses from inputs that arrive at those points too soon.
The myelin that has already formed on axons has to be thickened or thinned in a controlled way to speed or slow signal transmission. Prior to our findings, there was no known explanation for how the myelin sheath could be thinned to slow signals, aside from disease damage. Our research revealed another type of glial cell involved in these “plastic” nervous system changes.
Surrounding the node of Ranvier is a glial cell called an astrocyte. Astrocytes have many functions, but most neuroscientists have largely ignored them because they do not communicate with other cells through electrical impulses. Surprisingly, research in the past decade has shown that astrocytes positioned close to the synapse between two neurons can regulate synaptic transmission during learning by releasing or taking up neurotransmitters there. But until fairly recently, myelin biologists tended to ignore the unique type of astrocyte that contacts an axon at a node of Ranvier.
What exactly do these so-called perinodal astrocytes do to thin the myelin sheath? Just as one would begin when remodeling a garment, these cells assist in cutting the “seams.” The myelin sheath is attached to the axon by a spiral junction flanking the node of Ranvier. Under an electron microscope these junctions appear as spirals of stitches between the axon and the myelin, and the threads that form each stitch are composed of a complex of three cell adhesion molecules. Our analysis of the molecular composition of these stitch points showed that one of these molecules, neurofascin 155, has a site that can be cleaved by a specific enzyme, thrombin, to thin the myelin.
Thrombin is made by neurons, but it also can enter the brain from the vascular system. As the myelin lifts off the axon, the amount of bare axon at the node of Ranvier increases. The outer layer of myelin is attached to the axon adjacent to the perinodal astrocytes. When the myelin is detached from the axon, the outer layer withdraws into an oligodendrocyte, thinning the sheath. Both widening of the nodal gap and thinning of the myelin sheath slow the speed of impulse transmission.
We found that the enzyme’s snipping of these threads that stitch myelin to the axon can be controlled by the perinodal astrocyte’s release of an inhibitor of thrombin. We carried out experiments on genetically modified mice in which astrocytes released less of this thrombin inhibitor. When we looked at their neurons with an electron microscope, we could see that the myelin had thinned and that the nodal gap had increased. By using electronic amplifiers to detect neural impulses and measure their speed of transmission, we found that after the myelin thickness decreased in this way, the speed of impulse transmission in the optic nerve slowed by about 20 percent and the animals’ vision declined. We were able to reverse all these changes by injecting thrombin inhibitors, which are approved for treating vascular disorders.
Our experiments support a new hypothesis: the myelin sheath’s changes in thickness represent a new form of nervous system plasticity governed by the addition and subtraction of myelin. Additional layers of myelin are not added to axons as one would wrap tape around a wire, because this would tie the legs of the oligodendrocytes in knots. Instead new insulation is affixed through the construction of a new inner layer that spirals around the axon like a snake below the overlying myelin. Meanwhile the outer layer of myelin can be detached by the perinodal astrocyte to thin the sheath. The thickness of the myelin sheath is not fixed; instead it reflects a dynamic balance between the addition of layers next to the axon and removal of the outer layer under control of the astrocyte.
Brainy Waves
The optimal timing of action potentials at relay points is critical for strengthening synapses by adjusting their timing to allow them to fire together. But myelin plasticity can contribute to neural circuit function and learning in another way—by tuning the frequency of brain-wave oscillations. Not all neural activity in the brain arises from sensory inputs. Much of it takes place because of what goes on in the brain itself at both conscious and unconscious levels. This self-generated activity consists of oscillating waves of different frequencies that sweep through the brain, just as the vibration of a car engine at a certain speed will set different parts of the automobile rattling together at resonant frequencies.
These brain waves, or oscillations, are believed to be a key mechanism for coupling neurons across distant regions of the brain, which may be important for sorting and transmitting neural information. Oscillations, for example, tie together neural activity in the prefrontal cortex, which provides contextual meaning, and in the hippocampus (responsible for encoding spatial information). This oscillatory coupling enables a person to quickly recognize a familiar face at work, but it also makes it more difficult to identify the same co-worker in an unfamiliar place.
More important, the various stages of sleep, critical for storing long-term memories, can be identified by brain waves oscillating at different frequencies. Our experiences accumulated during the day are replayed during sleep and sorted for storage or deletion based on how they relate to other memories and emotions, which can mark them as potentially useful (or not) in the future. Appropriate brain-wave oscillations are believed to be pivotal in this process of memory consolidation. But the speed of impulse transmission is critical in synchronizing brain waves.
Just as two toddlers must precisely time their leg movements to drive the up-and-down motion of a teeter-totter, the transmission delays between two populations of oscillating neurons must be timed so that coupled neurons oscillate in synchrony across long distances in the brain. Myelin plasticity is important for brain waves because the proper conduction velocity is necessary to sustain oscillations that couple two regions of the brain at the same frequency.
This conclusion is based on mathematical modeling of the fundamental physics of wave propagation done by me, together with my NIH colleagues Sinisa Pajevic and Peter Basser. In 2020 a study by Patrick Steadman and his colleagues in Paul Frankland’s lab at the University of Toronto provided convincing experimental support for the idea. Using genetically modified mice in which myelination could be temporarily halted, the researchers found that the ability to learn to fear an unsafe environment and to remember safe locations depends on the formation of new myelin. Moreover, they found that in this type of learning, brain-wave activity during sleep becomes coupled between the hippocampus and the prefrontal cortex. The prevention of new myelin formation also weakened connections and resulted in a type of impaired recall often found in people who have difficulty associating fear after a traumatic event with the appropriate context.
Learning and performing any complex task involves the coordinated operation of many different neurons in diverse brain regions and requires that signals proceed through large neural networks at an optimal speed. The myelin sheath is crucial for optimal transmission, but people begin to lose myelin in the cerebral cortex in their senior years. This gradual degradation is one of the reasons for cognitive slowing and the increasing difficulty of learning new things as we age.
Consider how transmission delays disrupt long-distance communication by telephone. Similarly, lags in the brain can cause cognitive difficulties and disorganized thinking in individuals with psychological disorders such as schizophrenia. Indeed, differences in brain-wave oscillations are seen in many neurological and psychiatric disorders. Alzheimer’s disease, for instance, is associated with changes in white matter.
Drugs that control myelin production could provide new approaches to treating these problems. Indeed, Fei Wang and his colleagues at the Third Military Medical University in China, in collaboration with Jonah Chan of the University of California, San Francisco, reported in 2020 that the myelination-promoting drug clemastine given to mice with a gene deletion that impairs development of oligodendrocytes improved learning tested in a water maze. Because myelination is influenced by many forms of neural activity, a number of techniques—for example, cognitive training, neurofeedback and physical therapy—may be helpful in treating age-related cognitive decline and other disorders. A 2018 study of older adults by Jung-Hae Youn and his colleagues in South Korea indicated that 10 weeks of memory-training exercises increased recall. Brain imaging before and after training revealed increased integrity of white matter tracts connecting to the frontal lobe in the group of seniors who undertook the memory-training sessions.
These novel concepts have begun to change the way we think about how the brain works as a system. Myelin, long considered inert insulation on axons, is now seen as making a contribution to learning by controlling the speed at which signals travel along neural wiring. In venturing beyond the synapse, we are beginning to fill out the stick-figure skeleton of synaptic plasticity to create a fuller picture of what happens in our brain when we learn.