How do you think the dark matter problem is solved?” Vera C. Rubin urgently asked me, within minutes of being introduced at a 2009 Women in Astronomy conference. To this day, I can’t remember what I said in response. I was awestruck: the famed astronomer who had won the National Medal of Science for her work finding the first conclusive evidence for dark matter’s existence was asking me, a twentysomething Ph.D. student, for my opinion. I am certain that whatever I came up with was not very good because it was a problem that I had, until that moment, given no serious thought to. Until Rubin asked me my opinion, it had never occurred to me that I was entitled to have an opinion on the question at all.
If I disappointed her with my answer, she didn’t show it. Instead she asked me to sit down to lunch with her and some other women astronomers, including former NASA administrator Nancy Grace Roman. Rubin then proceeded to fangirl over Roman, who is often referred to as “the mother of the Hubble Space Telescope.” It was quite a moment for me, to watch an elderly woman who had uncovered one of the greatest scientific mysteries of our time excitedly introduce us to her own hero.
Rubin cemented her legacy in the 1960s, when she studied stars inside galaxies and found something odd: stars on the outskirts of galaxies were moving faster than they were supposed to, as if there was an invisible matter there contributing a gravitational pull. Her work echoed findings from galaxy cluster studies in the early 1930s by Fritz Zwicky, which had led him to suggest the existence of Dunkle Materie, German for “dark matter.” Throughout the 1970s Rubin and astronomer Kent Ford published data consistent with this conclusion, and by the early 1980s scientists were in widespread agreement that physics had a dark matter problem.
Most attempts to track down dark matter in the laboratory have fallen into three categories. So-called direct detection experiments look for evidence of dark matter particles interacting with particles of normal matter—for instance, the element xenon—through one of the nongravitational fundamental forces, the weak force, as well as through hypothesized new forces. Collider experiments, such as those at the Large Hadron Collider near Geneva, take the opposite approach, smashing two regular particles together with the hope of producing dark matter particles. Meanwhile “indirect detection” experiments look for evidence of dark matter interacting with itself, with the resulting collision producing observable particles.
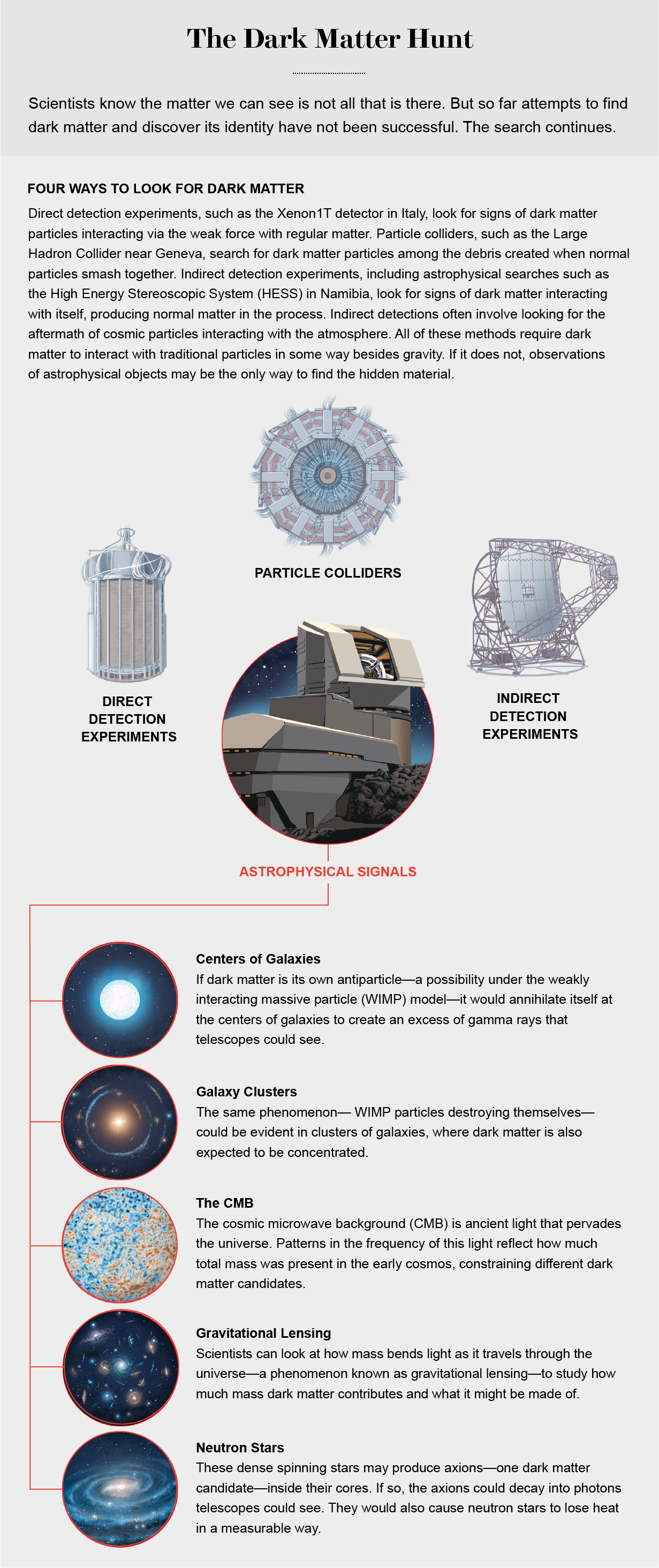
So far none of these strategies has turned up the missing matter. We still don’t know if dark matter can talk to regular matter in any way beyond gravity. It may be impossible to produce in the accelerators we can build or to detect in the experiments we can construct. For this reason, astronomical observations—cosmic probes of dark matter—are one of our best hopes. These probes allow us to look for signatures of dark matter in environments that are difficult for us to produce on Earth—for example, inside neutron stars. More broadly, such searches look at dark matter’s behavior under gravity in a variety of locations.
Despite the promise of this approach for studying dark matter, it has sometimes been caught in the middle between the astronomy and physics communities. Physicists tend to emphasize colliders and laboratory experiments and don’t always prioritize links to astrophysical work. Astronomers tend to write dark matter off as a particle physics problem. This disconnect has implications for funding. In 2022 we have an opportunity to change that. The start of the 2020s marked the beginning of an important process known as the Snowmass Particle Physics Community Planning Exercise. This project, which takes place about once a decade, brings physicists together to explain prospective scientific projects to a congressionally mandated panel that will determine scientific priorities. For the first time, cosmic probes of dark matter will be a distinct topic of consideration. Although Snowmass does not make formal policy recommendations, it is certainly the case that at each stage of the organizational hierarchy, there will be decisions about what science to emphasize.
A Universe of Dark Matter Candidates
There is still much we don’t know about dark matter, but we have come a long way since Rubin’s work in the 1970s and 1980s. We now have good evidence to suggest that every galaxy lives in its own bubble of dark matter—called a dark matter halo—that extends well beyond the visible part of the galaxy. The amount of dark matter in these galaxy-halo systems surpasses the amount of matter in the stars and planets and gas. In other words, all the particles that we have been able to identify in labs and colliders—referred to collectively as the Standard Model of particle physics—contribute only about 20 percent of the normally gravitating matter in the universe. If we take into account dark energy and the fact that matter and energy are fundamentally equivalent, we are down to understanding only about 4 percent of the cosmos. The Standard Model is both a stunning achievement and a theory that is, apparently, deeply incomplete. We need a new particle or particles to solve the problem.
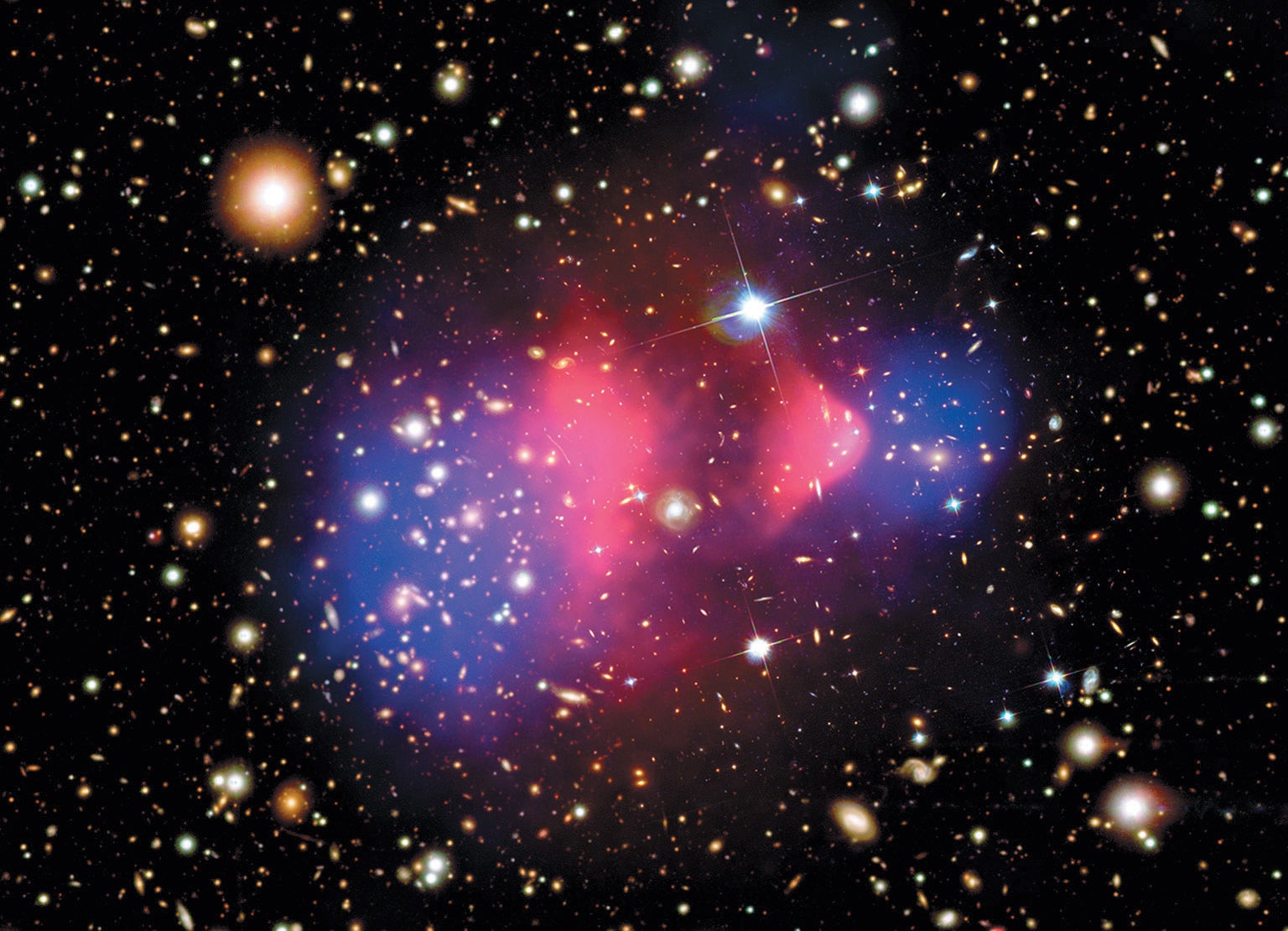
Physicists now have an assortment of dark matter candidates. Most scientists favor candidates that are “cold dark matter”—particles that move relatively slowly (meaning, at nonrelativistic speeds much slower than that of light). Within the class of cold dark matter, one of the classic models is the weakly interacting massive particle (WIMP). Scientists presume that WIMPs would have formed naturally in the early universe and predict that they have some kind of interaction with regular matter through the weak force. The most popular WIMP models fall into a category of particles called fermions—a class that includes electrons and quarks.
WIMPs were the most highly favored dark matter candidates for a long time, particularly in the U.S. Opinions have shifted in recent years, though, as evidence for WIMPs has failed to show up at the Large Hadron Collider or in any of the direct and indirect detection experiments.
Recently the particle physics community has become excited about another hypothetical dark matter candidate: an axion. Axions are predicted to have smaller masses than WIMPs, and they are not fermions. Instead axions belong to a class of particles called bosons—the category that includes photons, or particles of light. As bosons, axions have fundamentally different properties than WIMPs, which opens the door to an intriguing possibility about the structures they could form. Axions are what first drew me into the world of dark matter research.
Alluring Alternatives to WIMPs
Five years passed between my conversation with Vera Rubin and my first attempt at answering the question she had put to me. By then it was 2014, and I was a Dr. Martin Luther King, Jr., postdoctoral fellow at the Massachusetts Institute of Technology, appointed first to the Kavli Institute for Astrophysics and Space Research and then the Center for Theoretical Physics (CTP) and looking for something interesting to work on. It was there that Mark Hertzberg—at the time also a postdoctoral researcher at CTP—and I first started talking about a debate that had erupted among physicists: Could axions form an exotic state known from atomic physics called a Bose-Einstein condensate?
This possibility arises from a fundamental difference between bosons and fermions. Fermions must obey the Pauli exclusion principle, which means two fermions cannot share the same quantum state. This rule is why electron orbitals in chemistry can be so complicated: because the electrons orbiting an atom cannot occupy the same quantum state, they must spread out in different patterns with different amounts of energy called orbitals. Axions, on the other hand, can share a quantum state. This means that when we cool them enough they can all enter the same low-energy state and act collectively like one superparticle—a Bose-Einstein condensate. The possibility that this could happen naturally in space is, in my view, quite exciting.
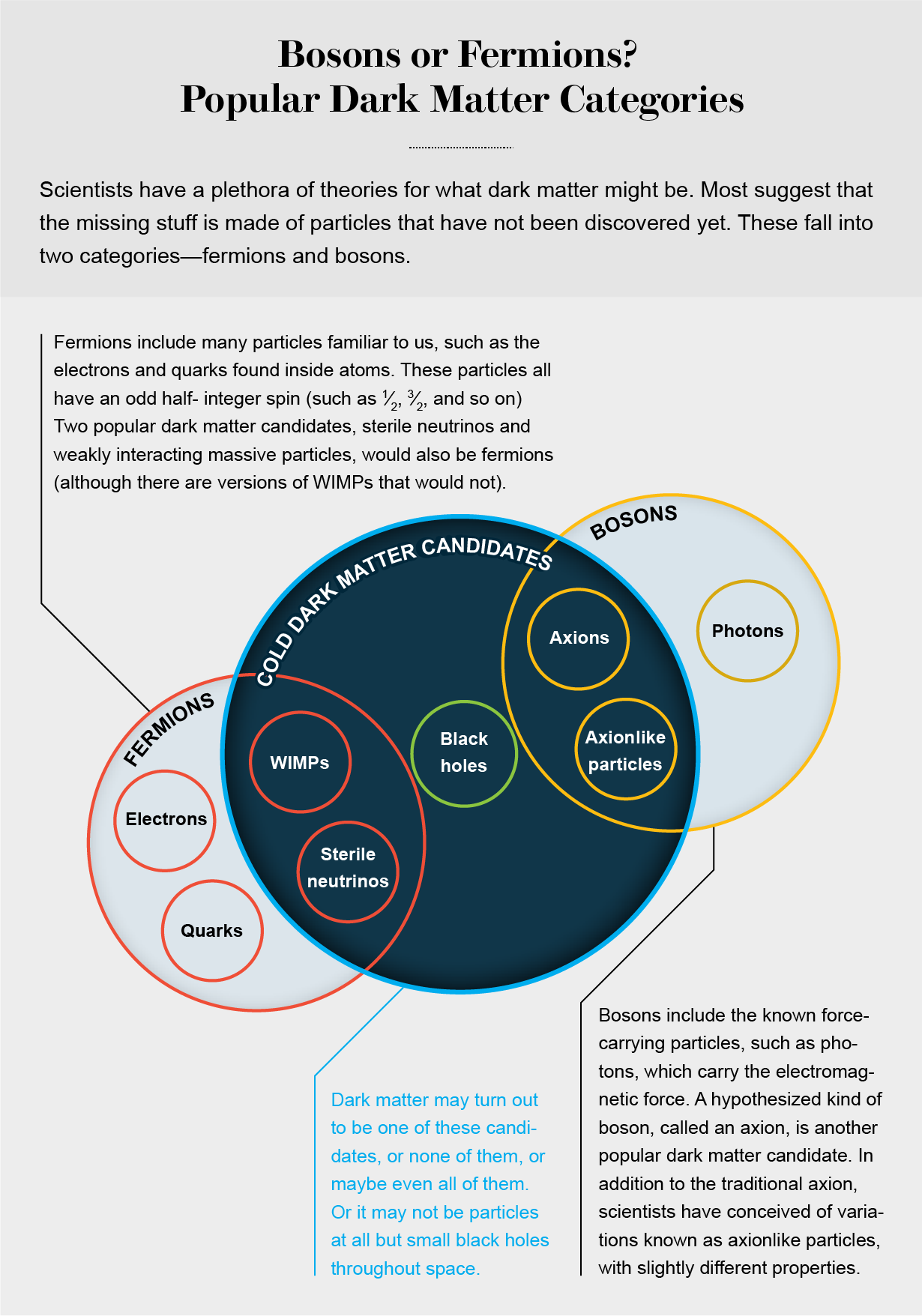
Axions had been proposed in the 1970s by Hertzberg’s Ph.D. adviser at M.I.T., Frank Wilczek, one of the first to realize that one consequence of a model proposed by Helen Quinn and the late Roberto Peccei was a particle, which Wilczek named “axion” after a brand of laundry detergent. Thus, Hertzberg was already quite familiar with axions. I, on the other hand, was relatively new to this idea. I had spent most of my career focused on other questions, and I had to get up to speed. Along the way, I learned to distinguish between the traditional axion and the class of particles that physicists have come to loosely refer to as axionlike particles.
The traditional axion arises from the Peccei-Quinn extension to the theory of quantum chromodynamics (QCD), which describes another of the four fundamental forces, the strong force. Although QCD is a highly successful model, it also predicts phenomena we’ve never observed. Peccei and Quinn’s work solves this problem, while providing a mechanism for producing dark matter. But another idea called string theory also proposes a series of particles with the same mathematical structure as the original axion; these particles have come to be called axionlike. The traditional QCD axion is usually expected to have a mass of about 10–35 kilogram—several orders of magnitude lighter than the electron—but the larger class of axions from string theory can be much lighter, down to 10–63 kilogram.
The work Hertzberg and I did together with our postdoctoral adviser Alan Guth led us to quibble with a popular view of how axions might form Bose-Einstein condensates. A distinguished physicist, Pierre Sikivie of the University of Florida, had prompted much excitement in 2009, when he proposed that QCD axions would form large condensates in the very early universe. His calculations suggested they would lead to ringlike galaxy halos rather than the spherical halos that most astronomers expect and that WIMP models predict. If so, then we might be able to tell what dark matter is made of just by looking at halo shapes.
But when Mark, Alan and I sat down to check how Sikivie’s group had arrived at this prediction, we came to a radically different conclusion. Although we agreed that axion Bose-Einstein condensates would form in the early universe, they would be much smaller—the size of asteroids. Our model also did not give any indications, in the present-day universe, of what kind of axion structures we might find billions of years in the future. Trying to better model how—and whether—we get from small asteroid-sized condensates to the galactic-scale dark matter halos of today is still a significant computational challenge.
The same year our paper came out, another group was looking into other interesting implications of axionlike particles. A team led by Hsi-Yu Schive of National Taiwan University published computer simulations of certain axionlike particles that are often referred to as “ultralight axions” or “fuzzy dark matter,” so named because they have a very low mass and would act like blurred-out waves rather than pointlike particles. They showed that these particles could form wavelike dark matter halos with Bose-Einstein condensates at their cores. Schive’s paper generated new interest in ultralight axions and raised hopes that astrophysical observations could detect signs of the wavelike halo structures we expect.
These days axions and axionlike particles stand along with WIMPs as some of our best guesses at what dark matter could be. Another category that is growing in popularity is a model called self-interacting dark matter (SIDM). This idea predicts fermion dark matter particles that have some kind of interaction with one another—a self-interaction—beyond gravity. These self-interactions could create more interesting shapes and structures within a halo than a smooth, spherical blob. The particulars of the structures are hard to predict, though, and depend on the mass and other characteristics of the particles. Interestingly, axions may also interact with one another, though in different ways than self-interacting fermions.
There is an alternative to WIMPs, axions and SIDM: neutrinos. Although Standard Model neutrinos are now known to be too low in mass to explain all of the missing matter, these neutrinos are real and hard to see, making them functionally a small component of the dark matter that we call the cosmic neutrino background. In addition, a new type of neutrino has been hypothesized as a companion to the Standard Model neutrino: the sterile neutrino. Sterile neutrinos are distinct because they interact primarily gravitationally and only mildly through Standard Model forces. In addition, they are perhaps the most popular warm—or at least somewhere between hot and cold—dark matter proposal.
Another idea that theorists are just starting to explore is that rather than a single dark matter particle, there may be an entire sector. Perhaps dark matter is made of traditional axions, axionlike particles, WIMPs, sterile neutrinos and SIDM—all together. One other tantalizing possibility is that dark matter actually comprises stellar-mass black holes that would have formed in the early universe. This option has become more popular since the 2017 detection of gravitational waves indicated that black holes in this mass range are more common than expected.
Clues in the Sky
In astronomy we are relatively passive observers. We can choose our instruments, but we cannot design a galaxy or a stellar process and watch it unfold. Cosmic phenomena rarely happen on human-friendly time scales—galaxy formation takes billions of years, and the cosmic processes that might emit dark matter particles do so over tens to hundreds of years.
Even so, astrophysical probes of dark matter can tell us a lot. For instance, the NASA Fermi Gamma-ray Space Telescope has functioned as a dark matter experiment by looking for gamma-ray signatures that could be explained only by dark matter. WIMPs, for instance, are predicted to be their own antimatter partners, meaning that if two WIMPs collided, they would annihilate each other just as matter and antimatter do on contact. These explosions should produce an abundance of gamma-ray light where there is dark matter, especially at the cores of galaxies where dark matter is densest.
In fact, the Fermi telescope does see an excess of gamma-ray light at the center of the Milky Way. These observations have inspired passionate debate among observers and theorists. One interpretation is that these fireworks result from dark matter colliding with itself. Another possibility is that the signal comes from neutron stars near the center of the Milky Way that emit gamma-ray light through the typical course of their lives. Some astrophysicists favor the more mundane neutron star explanation, but others think the signal is dark matter. The fact that there is disagreement is normal, and even I have a hard time deciding what I think. I am compelled by physicists Tracy Slatyer and Rebecca Leane’s thoughtful research showing that a dark matter explanation is sensible, but in the end, only analysis of more detailed observations will persuade the community about either idea. Future data from the Fermi telescope and proposed experiments such as NASA’s All-sky Medium Energy Gamma-ray Observatory eXplorer (AMEGO-X for short) have the potential to settle the debate.
Scientists have also used the Fermi telescope to look for evidence of axions. Theories predict that when axions encounter magnetic fields, they occasionally decay into photons. We hope that by looking over long distances, we might see signs of this light, offering proof that axions exist. And neutron stars—the potential confounding signal at the Milky Way’s center—are actually a good place to look for dark matter on their own. Some theories suggest that these dense spinning stars produce axions when protons and neutrons collide in their cores. We might be able to observe these axions as they decay into photons and escape from the stars. And as neutron stars release the dark matter over tens to hundreds of years, they would cool down in a pattern that we may be able to measure—if we look long enough. Another hot topic of study right now is whether nonaxion dark matter collects in neutron stars, affecting the structure of the star. As a member of the NASA Neutron Star Interior Composition Explorer (NICER) collaboration, I am leading a research project that is using data from NICER, a little telescope on the International Space Station that is up for renewal later this year. Our project is looking for evidence that dark matter is inside or enveloping neutron stars.
We can also learn more about the nature of dark matter by studying the best evidence we have for its existence so far—the cosmic microwave background (CMB) radiation. This light is a radio signal that originated in the early universe, and it is inescapably everywhere, all around us. It provides a snapshot of a moment early in cosmic history, and the patterns we see in the frequencies of its light reflect the makeup of the universe when it was created. It turns out that we can only explain the patterns we see in the CMB by assuming that dark matter was present—if there were no dark matter, the CMB data would make no sense. The patterns in the data tell us what fraction of the total mass and energy dark matter contributed; they even help constrain the possible masses of the dark matter particles. As I write, the CMB-Stage 4 collaboration is preparing to use a collection of telescopes in Chile’s Atacama Desert and at the South Pole to take the most detailed measurements yet of the CMB.
On the Horizon
Since that 2009 Women in Astronomy conference, Rubin and Roman have both passed away, but their legacies live on through projects that will seek to better understand our universe. The NASA Nancy Grace Roman Space Telescope will launch in the mid-2020s, and although it is primarily focused on studying cosmic acceleration (the “dark energy problem”) and exoplanets, it will also provide insight into dark matter. At the same time, here on Earth, the Vera C. Rubin Observatory in the Atacama Desert will support research on many questions, including the search for the dark matter that made Rubin famous.
In other words, we have lots to look forward to in the coming years. One reason is that almost any large-scale astronomical observation has something to tell us about dark matter. For example, a team in Mexico led in part by Alma X. Gonzalez-Morales and Luis Arturo Ureña-López showed that we can use the phenomenon of gravitational lensing, where large masses bend spacetime so much that it acts like a fun-house mirror, to place constraints on the mass of fuzzy dark matter. Gonzalez-Morales and Ureña-López are both active participants in the Rubin Observatory’s Legacy Survey of Space and Time program, working on gravitational lensing and participating in the dark matter working group. Within the group, we are discussing observations that will capture more detailed information about dark matter halos that can then be compared with computer simulations of proposed dark matter candidates. Similarly, Roman telescope surveys of large-scale structure will provide insight into dark matter’s behavior on cosmic scales.
In the future, proposed x-ray observatories such as the NASA Spectroscopic Time-Resolving Observatory for Broadband Energy X-rays (STROBE-X) can help us take a closer look at neutron star structure in ways that will enhance our understanding of dark matter’s possible properties. Other proposed future projects such as NASA’s All-sky Medium Energy Gamma-ray Observatory, or AMEGO (not to be mistaken for AMEGO-X), will do the same in a different wavelength.
I will be an active participant not just as a scientist but as one of three conveners, alongside Alex Drlica-Wagner and Hai-Bo Yu, for the Snowmass Cosmic Frontier’s topic Dark Matter: Cosmic Probes. It is our responsibility to describe the excitement and possibilities of astrophysical searches for dark matter to the funding decision-makers. The document I will help produce may influence guidance that is given to the National Science Foundation and the U.S. Department of Energy about what research we conduct over the next decade.
Coincidentally, the astronomy community just recently completed a similar process known as the 2020 Decadal Survey on Astronomy and Astrophysics. The resulting report sidestepped substantively addressing the dark matter problem, but it still offered strong support for efforts to better map the CMB, instruments to study neutron stars and x-ray observatories—three goals that will help us understand dark matter.
Doing science is never just about calculations, observations and experiments; it is also about working collaboratively with other people, including policy makers. How much progress we make will depend in part on what kind of support we get from lawmakers. Of course, this is stressful to think about. The good news is that there is a universe to wonder about, and trying to understand dark matter is a great distraction.